The towering heights and steep slopes of mountains make them some of the most striking geological features of our global landscapes and they have taken part in our myths and legends for millennia. How can we model the topography of these captivating landforms, and what do we understand about how they have come to be?
Orogenesis, building mountains
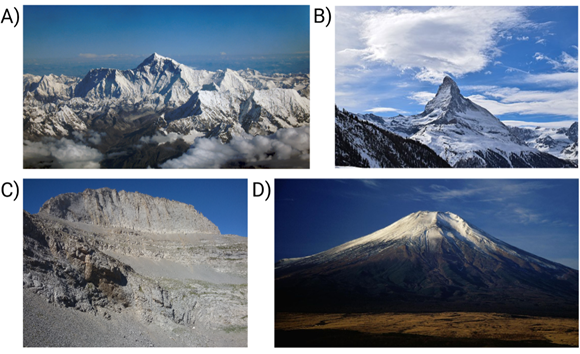
The process of orogenesis, mountain formation, generally takes place in regions of colliding tectonic plates known as subduction zones5. Tectonic plates consisting of older and denser lithosphere material – such as at the edges of spreading oceanic basins – tend to be involved in orogenesis. As two continental plates collide with each other, the subducted (lower) plate is dragged under the upper plate at its passive continental margin. This is driven by an attached oceanic slab, made up of relatively dense lithosphere, dragging the subducting plate further and further into the depths of the mantle. Under immense temperatures and pressure, both continental plates are deformed and fold up into nappes, typically coinciding with mass metamorphic events of their rocks. Powerful, long-term frictional forces eventually break off the oceanic slab from the subducted continental plate, known as slab breakoff, and the mass of the subducted plate is reduced. As a result, the subducted continental plate rises again via isostatic uplift (positive buoyancy) where it pushes thickened continental crust up at the surface, forming mountain chains (orogens). Imagine pushing a buoyant balloon underwater, then releasing and watching it rise upwards at the water’s surface. The splash at the surface of the water is analogous to the crustal uplift caused by slab breakoff in a subduction zone.
But if this process is common to the formation of all of Earth’s mountain ranges, then why do mountains in different regions appear so dissimilar? The ultimate mountain size and shape depends not only on the context of regional subduction zones, but also on the geological history, climate and erosional processes taking place. This is perhaps best illustrated by some of the most famous examples of mountains (Figure 1).
The Himalayas and Mount Everest
The Himalayas (Fig. 2) consists of a series of mountain ranges along a length of the south western border of China, spanning parts of Bhutan, India, Nepal and Pakistan5. Renowned for its barren landscape and extreme heights, the Great Himalayan range contains four mountains with an elevation over 8,000m (out of 14 such mountains globally). The Himalayas were formed by the classic Alpine style of orogeny which occurs when the expansion of an ocean basin coincides with continental break-up, ultimately leading to continent-continent plate collision. In this case, the closure of the Tethys Ocean during the Palaeozoic and Mesozoic, ultimately finishing 40 million years ago, coincided with collisions of portions of the supercontinent Gondwana with what is now Asia. The Himalayas formed as India collided with Asia around 55 million years ago, with the subduction of the Indian continental margin leading to deformation, crustal stacking and uplift. Part of the reason that the Himalayas contains so many of the world’s tallest mountains is that this subduction of the continental Indian plate formed the thickest crust found on Earth, producing massive peaks when this was eventually uplifted.
The tectonics of orogeny alone cannot explain the modern mountain forms of the Himalayas6,7. Owing to the climate of southern Asia, the Himalayas experience strong seasonal monsoon rains which ferociously erode the mountainsides and steepen the summits of these mountains. Erosion acts more strongly in the valleys than on the mountain summits, deepening the valleys and making the summits appear taller in relative height. In turn, this removal of crust also reduces the mass of the subducted continental margins in this region, allowing further uplift of the stacked crust which caused the Himalayas to continually grow in height over time. Recent estimates suggest that the Himalayas had a similar mean elevation in the Late Early Miocene as they do today, suggesting that these major alterations in mountain elevation did not occur in the last 9-15 million years8.
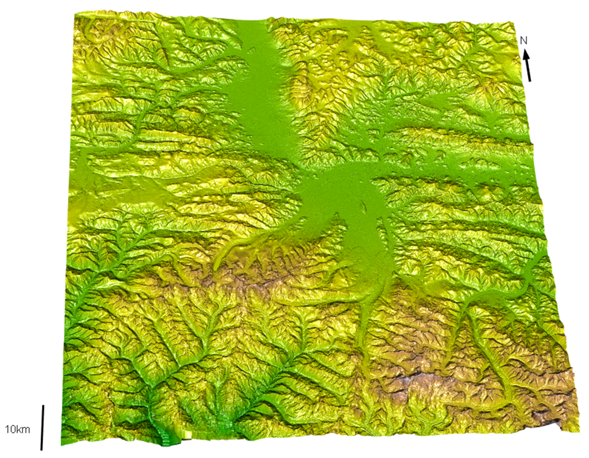
The most famous of the Himalayas is Mount Everest (Fig. 3), the highest mountain above sea level at 8,848m high8,10. The main summit pyramid of Mount Everest reveals part of the South Tibetan Detachment System (STDS), a series of faults and shear zones at the top of the Himalayan mid-crust which run all the way along the Himalayan orogen (2000km). The STDS illustrates the history of the Himalayas in a geological form. At the top of Mount Everest, the lower Lhotse separates lower Greater Himalayan sequence rocks (Fig. 3, green) from those of the Everest series above (Fig. 3, yellow), whilst the upper Qomolangma detachment lies within Tethyan sedimentary rocks and those metamorphosed during deformation (Fig. 3, grey and brown).
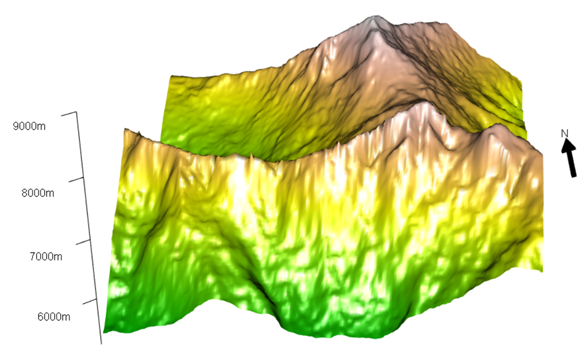
The Alps
The Alps are the major mountain range of Europe, forming an arc across the northern border of Italy and spilling over into neighbouring countries including France, Switzerland, Austria and Slovenia5,11. Although the Alps are another classic Alpine orogen, the orogenesis of the Alps is more complex than that of the Himalayas because it actually consists of two consecutive events. The older Mesozoic Eoalpine event formed the eastern Alps and later the Australoalpine nappes by expansion of the South Penninic Ocean and subduction and obduction of the Tethys Ocean. Then the more recent Cenozoic event led with the closure of the South Penninic Ocean. Importantly, this Cenozoic orogenesis event included the collision of the Australoalpine (African) realm with the Middle Penninic continental mass (Alpine Tethys) and then the European continental plate (Helvetic realm). These collisions formed a complicated stack from the top Australoalpine (eastern Alps), middle Penninic and lower Helvetic nappes (central and western Alps). The final uplift stage saw these stacked nappes being filled with sediments, known as flysch, further complicating the geology of the Alpine region.
The Swiss Alps and the Matterhorn
The Matterhorn is a very distinctive, horn-shaped mountain within the Zermatt region in Valais, Switzerland and straddling the Swiss-Italian border (Fig. 4)11. The Dent Blanche klippe containing the Matterhorn is an Australoalpine continental raft of African origin thrusted on top of the remains of the ancient Tethys Sea, with the European Helvetic plate held underneath. The Matterhorn is the quintessential example of a karling, a peaked mountain with steep faces and sharp edges that results from glaciers selectively eroding each face (Fig. 5). These glaciers carved out cirques via glacial abrasion which eventually met at the edges, forming the characteristic pyramidal shape of the karling.
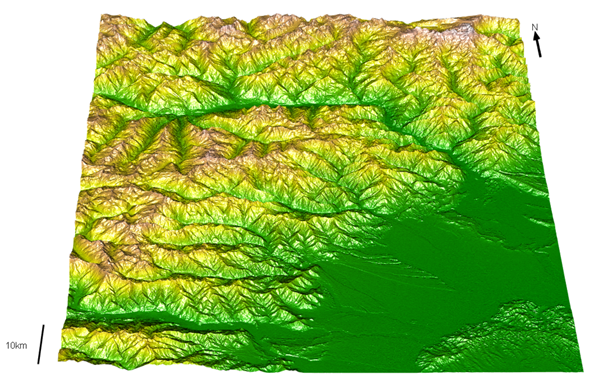
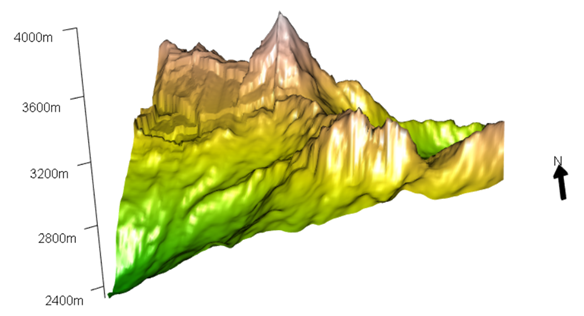
The Greek Alps and Mount Olympus
The Hellenic Alps of Greece (Fig. 6) also formed by collision of the Apulian plate with the European plate which led to the subduction of Apulian continental crust material12. As one might expect from a classic Alpine orogeny, deformation took place during this continent-continent plate collision and coinciding with closure of the Vardar ocean. Mount Olympus (Fig. 7) is the highest peak in Greece forming a impressive limestone block with a maximum elevation of 2,917m and is an important location in Greek mythology as the home of the Gods12,13,14. Originally these summit limestones were part of the lower, subducted continental plate and were only brought to the surface by normal faulting uplift. The exposed carbonate rocks are now structured in concentric layers around the base of Mount Olympus and so provide a tectonic window through the upper subducting rock layers. An interesting, yet obscure, connection has been made between the tectonic history of the Mount Olympus region and a lesser-known Greek mythology15. According to Apollodorus, Poseidon and Iphimedia had twin sons called Otus and Ephialtes (the Aloadae) which grew up with gigantism. As they grew older and larger, Otus and Ephialtes decided to climb Mount Olympus to fight the Gods for their right to marry two Goddesses who resided there. Together they constructed a geologic ladder by building Mount Ossa on top of Mount Olympus and then Mount Pelion onto Mount Ossa, which is consistent with the series of uplift events in the tectonic history of this region.
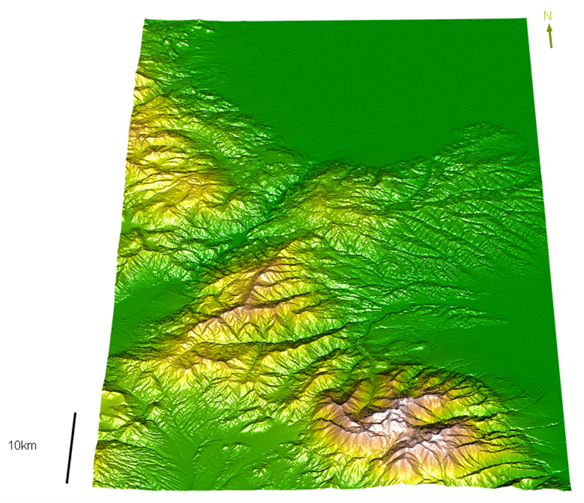
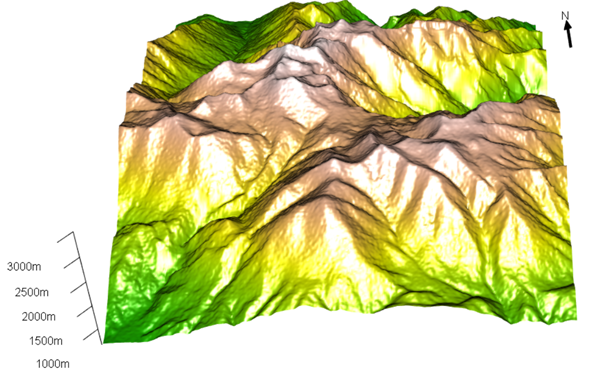
Mount Fuji, Japan
The very same subduction zones of colliding plates generate the volcanism typical of island arcs5. In the Japanese island arc, the Pacific Ocean plate is subducted under a margin of the Eurasian and North American continental plates and this interaction causes rising magma to collect within this subduction zone15. These plates detached from the Asian continental plate due to the spreading of the Sea of Japan. As the subducted oceanic crust sinks, water interacts with the mantle producing rising magma that collects into a magma chamber once it has reached gravitational equilibrium within the middle crust.
Mount Fuji is a young stratovolcano, with its typical steep slopes and cone shape, and reaches the highest elevation of any peak in Japan at 3,776m high (Fig. 8)17,18. Mount Fuji is unusual for a stratovolcano in its relatively frequent activity and basaltic ejecta, which is unfortunate given that it lies only 100km northwest of Tokyo. Although most know Mount Fuji as the main cone-shaped volcano, complete Fuji volcanism consists of several volcanic products from a much longer volcanic history. The oldest is Sen-Komitake, which started growing 400,000 years ago, and now lies underneath Komitake which can be seen on the exposed northern slope. Directly underneath the main cone lies Mount Shin-Fuji and Mount Ko-Fuji which have had active phases much more recently. The propensity for this region to form multiple volcanoes may relate to the significant depth that the Pacific Ocean plate slab is subducted underneath (170km) or alternatively Mount Fuji may hold two magma chambers which relay magma between them to maintain frequent eruptions over time. Despite the summit of Mount Fuji being inactive for 2,000 years there have been two recent eruptions from the flanks. The Jorgan eruption of 864-66 was slow and effusive, leaving lava flow on north-western flanks, in contrast to the more explosive Hoei eruption of 1707-08. The Hoei eruption covered much of Edo (Tokyo) with ashfall and left the Hoei craters on the southeast flank, which can still be seen today (Fig. 8, south east of the summit).
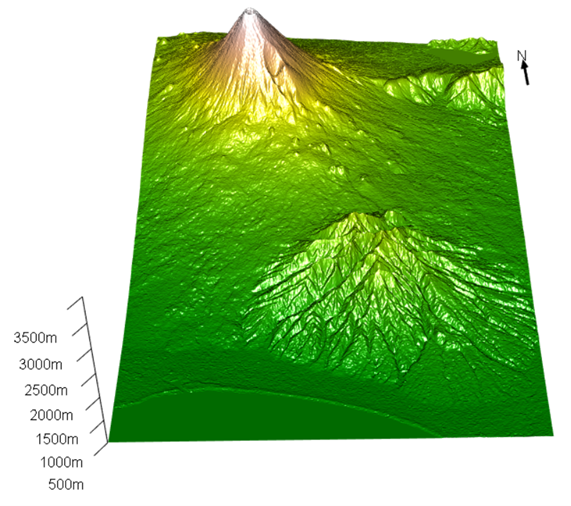
Method and Materials: 3D Topography Models
Firstly, I located the area of interest and downloaded the relevant 1-Arc Second Global Shuttle Radar Topography Mission (SRTM) data using the Earth Explorer application9. This global land elevation dataset was collected by the Endeavour shuttle with radar signals spaced at intervals of 1-arc second (~30m)19. I then converted the SRTM data to xyz coordinates using the conversion tool provided by QGIS software20. Next, I found the specific coordinates of the mountain of interest to be modelled using Open Street Map21 and generated the 3D model in R using the rgl package22.
References
- shrimpo1967 (2006) Mount Everest as seen from Drukair2. Wikipedia Commons. [Image Online] Available from: https://commons.wikimedia.org/wiki/File:Mount_Everest_as_seen_from_Drukair2.jpg
- Liridon (2019) Matterhorn, March 2019 (01). Wikipedia Commons. [Image Online] Available from: https://commons.wikimedia.org/wiki/File:Matterhorn,_March_2019_(01).jpg
- Diomidis Spinellis (2016) Stefani ridge at Mount Olympus. Wikipedia Commons. [Image Online] Available from: https://commons.wikimedia.org/wiki/File:Stefani_ridge_at_Mount_Olympus.jpg
- Alpsdake (1994) Mount Fuji from Hotel Mt Fuji 1994-11-29. Wikipedia Commons. [Image Online] Available from: https://commons.wikimedia.org/wiki/File:Mount_Fuji_from_Hotel_Mt_Fuji_1994-11-29.jpg
- Frisch, W., Meschede, M. and Blakey, RC. (2011) Plate Tectonics – Continental Drift and Mountain Building. 1st ed. New York, NY, USA: Springer.
- Gebelin A., Mulch, A., Teyssier, C., Jessup, MJ., Law, RD. and Brunel, M. (2013) The Miocene elevation of Mount Everest. Geology. [Online] 41(7), 799-802. Available from: https://pubs.geoscienceworld.org/gsa/geology/article-abstract/41/7/799/131318/The-Miocene-elevation-of-Mount-Everest
- Larson, KP., Graziana, R., Cottle, JM., Apen, F., Corthouts, T. and Lageson, D. (2020) The structural evolution of the Qomolangma Formation, Mount Everest, Nepal. Journal of Structural Geology. [Online] 138, 104123. Available from: https://www.sciencedirect.com/science/article/pii/S0191814120300985?via%3Dihub
- Kellett, DA., Cottle, J. and Larson, K. (2019) The South Tibetan Detachment System: history, advances, definition and future directions. Geological Society London Special Publications. [Online] 483(1), SP483.2. Available from: https://www.semanticscholar.org/paper/The-South-Tibetan-Detachment-System%3A-history%2C-and-Kellett-Cottle/258359994bc17c6dd5836fdf1e7a9cf8cbb40217
- (2021) EarthExplorer. USGS, science for a changing world. [Website] Available from: https://earthexplorer.usgs.gov/
- Pfiffner, AO. (2021) ‘The Geology of Switzerland’ in Reynard, E. (ed) Landscapes and Landforms of Switzerland. 1st ed. Basel, Switzerland: Springer International Publishing, pp. 7-30.
- Marthaler, M. and Rougier, H. (2021) ‘An Outstanding Mountain: The Matterhorn. Landscapes and Landforms of Switzerland’ in Reynard, E. (ed) Landscapes and Landforms of Switzerland. 1st ed. Basel, Switzerland: Springer International Publishing, pp. 187-199.
- Schermer, ER., Lux, DR. and Burchfiel, BC. (1990) Temperature-time history of subducted continental-crust, Mount Olympos Region, Greece. Tectonics. [Online] 9(5), 1165-1195. Available from: https://agupubs.onlinelibrary.wiley.com/doi/abs/10.1029/TC009i005p01165
- Smith, GW., Nance, RD. and Genes, AN. (1997) Quaternary glacial history of Mount Olympus, Greece. Geological Society of America Bulletin. [Online] 109(7), 809-824. Available from: https://www.researchgate.net/publication/233934044_Quaternary_glacial_history_of_Mount_Olympus_Greece
- Nance, RD. (2010) Neogene – Recent extension on the eastern flank of Mount Olympus, Greece. Tectonophysics. [Online] 488(1-4), 282-292. Available from: https://www.sciencedirect.com/science/article/pii/S0040195109002911
- Mariolakos, ID. and Manoutsoglou, E. (2013) The Geotectonic Evolution of Olympus Mt. and Its Mythological Analogue. Bulletin of the Geological Society of Greece. [Online] 47(2), 574-581. Available from: https://ejournals.epublishing.ekt.gr/index.php/geosociety/article/view/11084
- Nakada, S. (2018) ‘Volcanic Archipelago: Volcanism as a Geoheritage Characteristic of Japan’ in Chakraborty, A., Mokudai, K., Cooper, M., Watanabe, M. and Chakraborty, S. (eds) Natural Heritage of Japan – Geological, Geomorphological and Ecological Aspects. 1st ed. Basel, Switzerland: Springer International Publishing, pp. 19-28.
- Chakraborty, A. and Jones, TE. (2018) ‘Mount Fuji: The Volcano, the Heritage, and the Mountain’ in Chakraborty, A., Mokudai, K., Cooper, M., Watanabe, M. and Chakraborty, S. (eds) Natural Heritage of Japan – Geological, Geomorphological and Ecological Aspects. 1st ed. Basel, Switzerland: Springer International Publishing, pp. 167-176.
- Aoki, Y., Tsunematsu, K. and Yoshimoto, M. (2019) Recent progress of geophysical and geological studies of Mt. Fuji Volcano, Japan. Earth-Science Reviews. [Online] 194, 264-282. Available from: https://www.sciencedirect.com/science/article/pii/S0012825218302046
- (2021) USGS EROS Archive – Digital Elevation – Shuttle Radar Topography Mission (SRTM) 1 Arc-Second Global. USGS, science for a changing world. [Website] Available from: https://www.usgs.gov/centers/eros/science/usgs-eros-archive-digital-elevation-shuttle-radar-topography-mission-srtm-1-arc?qt-science_center_objects=0#qt-science_center_objects
- (2021) QGIS. A Free and Open Source Geographic Information System. [Software] Version 3.20.3, open source.
- Coast, S. (2021) OpenStreetMap. [Website] Available from: https://www.openstreetmap.org/#map=9/27.8998/86.6107
- (2021) rgl: 3D Visualization Using Open GL. The Comprehensive R Archive Network. [Website] Available from: https://cran.r-project.org/web/packages/rgl/index.html